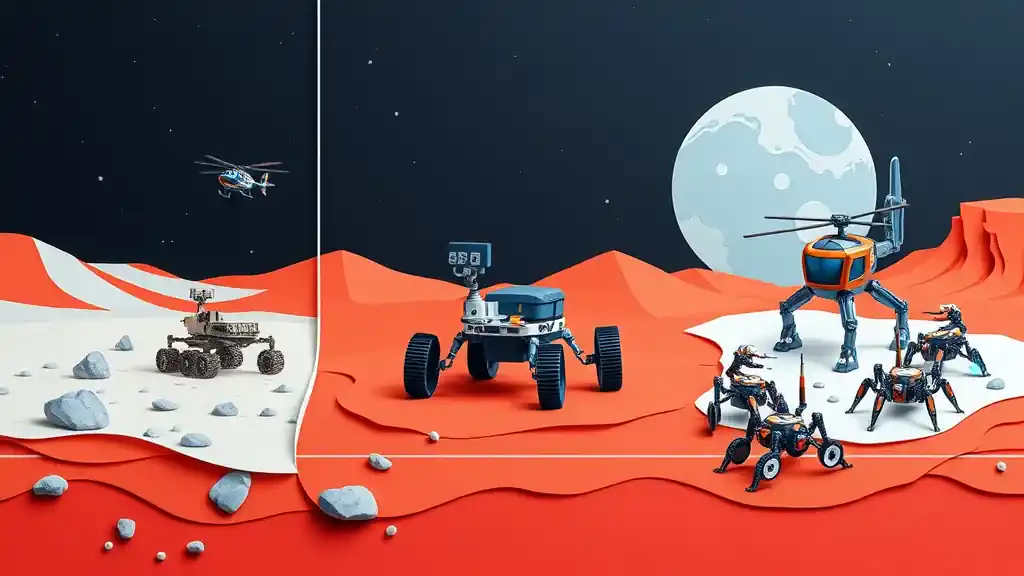
Space Robotics: From Simple Tools to Autonomous Explorers
system Collection
Key Insight
Introduction
State of the Art
Complication
1. Past: The Evolution of Space Robotics

When the Soviet Luna 9 made the first soft landing on another world in 1966, its primitive systems represented humanity's first robotic emissary on alien soil. From these humble beginnings—with computers less powerful than a modern calculator—emerged the foundation of space robotics that would eventually allow us to touch the surface of Mars, sample distant asteroids, and peer into the atmospheres of distant planets.
1.1 Subsystem Level: Early Robotic Components and Technologies
The earliest space robotic components emerged from necessity rather than ambition. In the 1960s and 70s, aerospace engineers faced the fundamental challenge of creating materials that could survive in space while meeting strict mass constraints. This led to pioneering work in radiation-hardened electronics and specialized lightweight alloys that formed the foundation of space robotics.
Sensing technologies started remarkably simple. The first space-based sensors were little more than basic photocells and primitive cameras. The Viking landers of 1976 🌐 represented a quantum leap forward, featuring what was then considered advanced visual processing capabilities—though by today’s standards, they’d be outperformed by a budget smartphone.
Early manipulator systems were mechanical marvels despite their limitations:
- Minimal degrees of freedom (typically 2-3)
- Direct teleoperation rather than autonomous function
- Simple end effectors with basic gripping capabilities
- Mechanical rather than electronic feedback systems
Power generation represented another critical challenge. Early robotic missions relied on:
Power System | Advantages | Limitations |
---|---|---|
RTGs (Radioisotope Thermoelectric Generators) 🌐 | Long-lasting, reliable | Limited power density, safety concerns |
Solar panels | Renewable, scalable | Dependent on sunlight, degradation issues |
{.smallt} |
Communication hardware utilized rudimentary radio frequency technologies with bandwidth measured in bits rather than megabits per second. This necessitated extremely efficient data compression—engineers had to make every bit count.
Thermal control initially relied on passive systems using materials with specific thermal properties. Without active regulation, operations were severely constrained in extreme temperature environments.
Perhaps most limiting was computational capability. Onboard processing was measured in kilobytes, forcing most complex operations to be Earth-controlled. The Apollo Guidance Computer, considered advanced for its time, operated with approximately 74 kilobytes of memory 🌐 —less than what’s needed to store a single modern digital photo.
1.2 System Level: Pioneer Robotic Explorers and Their Capabilities
The first true robotic explorers of space emerged in the 1960s and 70s, establishing the foundation for all future space robotics. These pioneering systems balanced rudimentary autonomy with Earth-based control to achieve remarkable firsts.
The Soviet Luna 9 🌐 made history in February 1966 as the first spacecraft to achieve a soft landing on another world. After landing on the Moon, its petals opened automatically to stabilize the craft, allowing its camera to capture and transmit the first images from the lunar surface. Though simple by today’s standards, this represented a breakthrough in automated space systems.
NASA’s Surveyor program 🌐 quickly followed with increasingly sophisticated capabilities:
Mission | Year | Key Robotic Capabilities |
---|---|---|
Surveyor 1 | 1966 | Autonomous landing sequence, remote-controlled camera |
Surveyor 3 | 1967 | First robotic soil sampling scoop, surface manipulation |
Surveyor 7 | 1968 | Advanced surface analysis tools, improved remote control |
The Soviet Lunokhod rovers marked a quantum leap forward. Lunokhod 1 🌐 (1970) operated for 11 months, traveling 10.5 km across the lunar surface. Despite being primarily teleoperated from Earth with significant communication delays, these rovers included rudimentary autonomous safety systems to prevent dangerous operations.
Viking landers (1976) represented the first Mars surface missions and incorporated true decision-making capabilities. Their sampling arms could select targets and adjust operations based on environmental readings—critical given the 20+ minute communication delay with Earth.
The Space Shuttle’s Remote Manipulator System (Canadarm) 🌐 introduced precision robotics to human spaceflight in 1981. This 15-meter robotic arm could deploy and retrieve satellites with remarkable accuracy despite operating in the challenging microgravity environment.
These pioneering systems established fundamental patterns still evident in modern space robotics: the careful balance between Earth control and onboard autonomy, redundant safety systems, and the critical importance of mass and power efficiency in robotic design.
1.3 Supersystem Level: Early Space Exploration Ecosystem and Infrastructure
The Cold War rivalry between the United States and Soviet Union created the perfect storm for robotic space exploration. National prestige wasn’t just at stake—it was the primary currency that unlocked unprecedented government funding. This geopolitical tension, while limiting international cooperation, paradoxically accelerated technological development as each superpower raced to claim “firsts” in space.
NASA’s establishment of the Deep Space Network (DSN) 🌐 in the 1960s represented a critical infrastructure investment that remains foundational today. These massive radio antenna complexes—strategically positioned approximately 120 degrees apart in California, Spain, and Australia 🌐 —ensured continuous communication with distant spacecraft regardless of Earth’s rotation. Without this global network, early robotic explorers would have been effectively blind and mute once they left Earth’s immediate vicinity.
Despite Cold War barriers, scientific communities gradually built bridges through organizations like COSPAR 🌐 (Committee on Space Research), established in 1958. These forums allowed limited data sharing while maintaining political separation.
The knowledge ecosystem supporting early space robotics relied on a complex partnership network:
Partner Type | Primary Contribution | Example |
---|---|---|
Universities | Theoretical foundations | MIT Instrumentation Lab |
National Labs | Applied research | Jet Propulsion Laboratory |
Aerospace Contractors | Hardware implementation | Hughes Aircraft Company |
Military Entities | Launch capabilities | US Air Force |
Public engagement played a surprisingly important role in sustaining political will for these expensive endeavors. Images from the lunar surface and distant planets captured global imagination despite limited media distribution channels. The famous “Earthrise” photo from Apollo 8 🌐 —though from a human mission—demonstrated how space imagery could transform public perception.
Early knowledge management relied primarily on paper documentation, with mission data stored in physical archives. This created significant limitations in cross-mission learning compared to today’s digital systems. Engineers often needed to “reinvent the wheel” due to information accessibility challenges.
Key Takeaways
- Early space robotics evolved from basic components in the 1960s-70s with severe limitations in computing power, energy capacity, and autonomous capabilities, requiring most operations to be Earth-controlled.
- Pioneer robotic explorers like Luna 9, Surveyor program, Lunokhod rovers, and Viking landers established fundamental design patterns balancing Earth-control with limited autonomous functions.
- The Cold War space race provided the geopolitical context and funding for early robotic exploration, while infrastructure like NASA's Deep Space Network created the foundation for communicating with distant robotic explorers.
- Despite technological constraints, these early missions established the engineering principles and operational frameworks that would influence decades of space robotics development.
2. Present: Current State of Space Robotics

While the Apollo astronauts relied on primitive onboard computers with less processing power than a modern calculator, today's space robots are technological marvels capable of autonomous exploration across the solar system. From Perseverance's ability to select its own science targets on Mars to the ISS's robotic arms performing delicate orbital surgery, we've entered an era where machines extend humanity's reach into the cosmos with unprecedented capability and independence.
2.1 Subsystem Level: Advanced Components Enabling Modern Space Robotics
The backbone of today’s space robotics lies in its advanced components—technological marvels that enable machines to thrive in the harshest environment known to humanity. These subsystems represent quantum leaps beyond their predecessors.
Computing Systems Modern space-grade processors deliver thousands of times more processing power than early missions while maintaining radiation hardening. The RAD750 processor 🌐 family, for example, can withstand radiation doses up to 1,000 times fatal human levels while performing complex onboard decision-making that would have been impossible just decades ago.
Materials Revolution Today’s robotic explorers utilize 🌐 :
Material Type | Key Features |
---|---|
Carbon Fiber Composites | Exceptional strength-to-weight ratios |
Ceramic Matrix Composites | Withstand extreme temperature variations |
Specialized Metal Alloys | Resistant to space radiation and atomic oxygen |
Integrated Sensing Sensor fusion technologies now combine data from multiple instruments:
- Visual, infrared, and multispectral cameras 🌐
- Spectrometers 🌐 and radiation detectors 🌐
- Environmental and tactile sensors 🌐
This integration creates comprehensive situational awareness for autonomous operations, enabling robots to “understand” their environment in ways impossible for single-sensor systems.
Scientific Instrumentation The miniaturization revolution has transformed bulky instruments into compact, multi-functional packages. Mars rovers now carry entire laboratories that would have required dedicated facilities previously. Perseverance’s SHERLOC instrument 🌐 , for instance, combines Raman spectroscopy and fluorescence analysis in a package smaller than a shoebox.
Power Systems Triple-junction solar cells now exceed 30% efficiency 🌐 , while lithium-ion batteries with specialized formulations provide reliable energy storage in extreme temperatures. Advanced power management systems dynamically allocate resources based on mission priorities.
Precision Actuators Modern space actuators feature:
- Embedded sensors 🌐 for position feedback
- Adaptive control 🌐 capabilities
- Dust-resistant designs 🌐 for planetary exploration
- Precision sufficient for sample handling and delicate operations 🌐
Communication Subsystems 🌐 Data transmission rates have increased by orders of magnitude through:
- Advanced signal processing algorithms
- Higher frequency bands utilization
- Sophisticated error correction protocols
These components work in concert, creating robotic systems far more capable than the sum of their parts.
2.2 System Level: Contemporary Robotic Explorers and Operational Frameworks
Today’s space robotics landscape represents a remarkable evolution in capability, autonomy, and operational sophistication. NASA’s Mars Perseverance rover stands as the pinnacle of planetary exploration robotics, featuring autonomous navigation systems that can identify and avoid hazards without Earth-based input. Its sample caching system—designed to collect and store Martian soil samples for future return—demonstrates unprecedented mechanical precision, while the accompanying Ingenuity helicopter has revolutionized our approach to planetary mobility.
In Earth orbit, the International Space Station’s robotic systems showcase different but equally impressive capabilities. Canadarm2 🌐 and Dextre 🌐 perform delicate maintenance tasks and capture incoming spacecraft with millimeter precision. These systems operate in a semi-autonomous mode where ground controllers can delegate routine operations while maintaining oversight for critical maneuvers.
The commercial sector has rapidly advanced space robotics through platforms like:
- Astrobotic’s Peregrine lunar lander 🌐
- Intuitive Machines’ Nova-C 🌐
- Maxar’s on-orbit servicing vehicles 🌐
These systems reflect a fundamental shift toward service-based operational models rather than purely scientific exploration.
Modern mission architectures increasingly incorporate multi-robot cooperation frameworks. The Mars Sample Return 🌐 mission exemplifies this approach, requiring coordinated operations between:
System Component | Primary Function | Autonomy Level |
---|---|---|
Sample Caching Rover | Collection and storage | High |
Sample Retrieval Lander | Transfer to ascent vehicle | Medium |
Orbital Return Vehicle | Earth transit | Medium-High |
Digital twin technology 🌐 creates high-fidelity virtual replicas that run parallel to physical systems, continuously synchronizing with actual hardware data. These sophisticated models enable engineers to detect anomalies when predicted and actual performance diverge, predict maintenance needs before failures occur, and safely test operational scenarios in a risk-free environment. This capability is particularly valuable for remote systems like Mars rovers, where engineers can validate changes virtually before implementing them on hardware that cannot be directly accessed.
The industry has also embraced standardized interfaces and modular design approaches. The International Docking System Standard 🌐 and the Robotic Refueling Mission 🌐 demonstrate how interoperability between different manufacturers’ systems has become essential for sustainable space operations.
Current operational procedures implement sliding autonomy models—robots handle routine operations independently but can request human assistance when encountering complex or unexpected situations. This balance maximizes efficiency while maintaining safety margins for mission-critical operations.
2.3 Supersystem Level: The Modern Space Robotics Ecosystem
The modern space robotics ecosystem has evolved into a complex network of interdependent stakeholders, technologies, and frameworks. Traditional space agencies like NASA, ESA, JAXA, and Roscosmos now share the stage with ambitious newcomers such as ISRO and CNSA, while commercial players like SpaceX and Blue Origin have dramatically reshaped the landscape.
International collaboration has become fundamental to advanced missions. The ExoMars rover represents a sophisticated project that was originally developed as an ESA/Roscosmos partnership (Following geopolitical developments related to Ukraine in 2022, ESA announced the suspension of the partnership). The upcoming Mars Sample Return mission demonstrates NASA and ESA’s integrated approach to solving complex engineering challenges. These collaborations distribute both technological risks and financial burdens across multiple nations.
The regulatory environment struggles to keep pace with innovation. The UN Committee on Peaceful Uses of Outer Space ( COPUOS 🌐 ) works to establish frameworks addressing critical concerns:
- Orbital debris mitigation protocols
- Planetary protection requirements
- Commercial activity boundaries
- Resource utilization rights
Global supply chains have created significant interdependencies despite geopolitical tensions. A typical space robot might include:
Component | Key Considerations |
---|---|
Radiation-hardened processors | Export control restrictions |
Advanced actuators | Precision manufacturing |
Composite structures | Quality certification challenges |
Vision systems | Dual-use technology concerns |
{.smallt} |
Public-private partnerships have transformed funding models, with government agencies increasingly acting as customers rather than operators. NASA’s CLPS program exemplifies this shift, purchasing lunar delivery services rather than building landers directly.
Space robots have also gained cultural significance through improved media coverage and direct public engagement. The Perseverance rover’s Twitter account 🌐 reaches millions, while citizen science initiatives allow direct participation in analyzing robotic mission data.
Key Takeaways
- Modern space robotics feature dramatic advancements in core technologies including radiation-hardened computing thousands of times more powerful than early missions, specialized materials with exceptional strength-to-weight ratios, and sophisticated sensor fusion systems enabling true autonomy.
- Current robotic explorers like NASA's Perseverance rover and the ISS's Canadarm2 demonstrate the pinnacle of contemporary capabilities, with multi-robot cooperation and digital twin technologies becoming standard operational approaches.
- Today's space robotics ecosystem has evolved into a complex landscape of international collaborations, public-private partnerships, and specialized supply chains, with diverse stakeholders from traditional space agencies to commercial entities reshaping how robotic missions are funded and operated.
- The cultural and educational impact of space robotics has expanded dramatically through improved media coverage, specialized academic programs, and direct public engagement initiatives that build support for continued exploration.
3. Future: The Trajectory of Space Robotics Development 🔮

As quantum computing merges with AI and self-healing materials, the robots exploring distant worlds tomorrow will bear little resemblance to today's machines. Imagine swarms of soft-bodied robots constructing habitats on Mars while subsurface explorers drill through Europa's ice to search for alien life—all operating with unprecedented autonomy across an emerging interplanetary internet that spans our solar system.
3.1 Subsystem Level: Emerging Technologies Reshaping Space Robotics
The next decade of space robotics will be defined by revolutionary subsystem technologies that dramatically expand capabilities beyond today’s constraints.
Quantum computing 🌐 represents perhaps the most transformative advancement, offering computational power orders of magnitude beyond current systems. This will enable:
- Real-time processing of complex scientific data
- Sophisticated autonomous decision-making
- Optimization of mission parameters without Earth-based intervention
Advanced AI systems 🌐 are evolving from narrow task-specific algorithms toward more general problem-solving capabilities. Future space robots will feature:
- Adaptive learning systems that improve performance during missions
- Cognitive architectures capable of handling unprecedented situations
- Collaborative multi-robot systems with distributed intelligence
Self-healing materials 🌐 incorporating biomimetic properties will fundamentally change how we approach spacecraft durability:
- Embedded sensor networks that detect microdamage before failure
- Active repair mechanisms using microencapsulated healing agents
- Programmable materials that can reconfigure to adapt to changing conditions
Neuromorphic computing architectures 🌐 modeled on biological neural systems offer dramatic efficiency improvements:
- Dramatic reduction in power requirements for equivalent processing
- Enhanced pattern recognition in noisy environments
- Improved sensor fusion and environmental understanding
Soft robotics using flexible materials and variable stiffness mechanisms 🌐 will create more versatile manipulation systems:
- Grippers that conform to irregular objects of unknown composition
- Locomotion systems adaptable to varied gravitational environments
- Damage-resistant structures that can operate after significant deformation
Advanced power systems will address the energy limitations that currently constrain robotic capabilities:
- Kilopower fission reactors 🌐 providing 1-10 kW for extended operations
- Next-generation radioisotope systems 🌐 with improved efficiency
- Wireless power transmission 🌐 for distributed robotic networks
Perhaps most revolutionary is the development of molecular manufacturing and nanoscale robotics 🌐 , which may eventually enable self-replicating systems that can construct complex structures from raw materials found in space.
3.2 System Level: Next-Generation Robotic Explorers and Architectures
The next decade will transform space exploration through revolutionary robotic architectures that fundamentally change how we explore and utilize space. These systems represent a paradigm shift from the single-platform approach that has dominated space exploration.
Swarm Robotics 🌐 : Strength in Numbers
- Deployments of 50-100+ specialized small robots working cooperatively
- Enables parallel operations across wide areas (particularly valuable for asteroid mapping)
- Provides built-in redundancy—mission continues even if individual units fail
- NASA’s Shapeshifter concept demonstrates this approach for Titan exploration
Human-Robotic Partnerships 🌐 Robotic systems will increasingly serve as force multipliers for human explorers:
- Precursor missions establishing infrastructure before human arrival
- Robotic assistants working alongside astronauts (GITAI’s robotic arm on ISS)
- Teleoperated robots extending human reach while minimizing risk
Autonomous Construction Systems 🌐 The transition from experimental to operational status is underway:
- 3D printing robots using regolith for habitats (AI SpaceFactory’s MARSHA)
- Autonomous landing pad construction robots (ESA’s PERIOD project)
- Self-replicating systems for exponential infrastructure growth
- Specialized drilling robots for Mars subsurface access
- Cryobots designed to melt through Europa’s ice shell
- Autonomous submarines for exploring subsurface oceans
Atmospheric Platforms
- Venus vehicle with extended duration capabilities 🌐
- Titan aircraft utilizing the dense atmosphere ( Dragonfly mission 🌐 )
- Hybrid systems combining multiple mobility modes
Sample Return Architectures
- Multi-stage missions with specialized collection and return vehicles
- Outer planet sample return missions with decade-long timelines
- On-site analysis paired with selective Earth return
Commercial Space Robotics
- Resource extraction systems for lunar regolith processing
- On-orbit manufacturing and assembly platforms
- Satellite servicing and orbital debris removal fleets
3.3 Supersystem Level: The Emerging Interplanetary Robotic Infrastructure
We’re witnessing the birth of a true interplanetary infrastructure that will fundamentally reshape our relationship with the solar system. This isn’t just about individual robots or missions anymore—it’s about creating a cohesive, distributed network that spans multiple worlds.
The emerging “Solar System Internet” represents perhaps the most critical foundation. NASA’s Delay/Disruption Tolerant Networking 🌐 protocols are already being implemented, with relay satellites around Mars forming the first nodes of what will become an interplanetary communication backbone. By 2040, we’ll likely see a network of communication satellites in strategic heliocentric orbits, enabling continuous high-bandwidth connections between Earth, the Moon, Mars, and beyond.
This infrastructure is developing within evolving governance frameworks. The 2020s have seen the first serious international discussions about autonomous robotic resource utilization, with the Artemis Accords representing an initial, though incomplete, approach. More comprehensive frameworks will be needed as robots begin making consequential decisions beyond real-time human control.
The public-private ecosystem supporting this infrastructure is maturing rapidly:
Sector | Current Focus | Emerging Trend |
---|---|---|
Commercial | LEO services, lunar landers | Specialized component providers |
Government | Deep space exploration | Infrastructure establishment |
Academic | Algorithm development | Human-robot interaction models |
Perhaps most exciting is the cross-pollination between Earth and space robotics. Technologies developed for disaster response robots are finding applications in lunar construction, while autonomous navigation systems designed for Mars rovers are being adapted for self-driving vehicles on Earth 🌐 .
This emerging infrastructure isn’t just about technology—it’s reshaping education and culture. Universities are already developing new curricula focused on robot fleet management rather than individual teleoperation, preparing a workforce that will oversee rather than directly control our mechanical emissaries.
Key Takeaways
- Next-generation space robotics will integrate quantum computing, advanced AI, and self-healing materials to enable unprecedented autonomy and durability in harsh space environments.
- Swarm robotics and hybrid human-robotic architectures will revolutionize exploration capabilities, with specialized robots working cooperatively on tasks from asteroid mining to constructing habitats using in-situ resources.
- Subsurface exploration systems and atmospheric platforms will access previously unreachable environments like Europa's subsurface oceans and Titan's atmosphere, dramatically expanding our search for extraterrestrial life.
- An emerging interplanetary infrastructure including communication networks, governance frameworks, and public-private ecosystems will form the foundation for humanity's expansion throughout the solar system.
Conclusion
From the primitive robotic explorers of the 1960s to today’s autonomous Mars rovers and tomorrow’s self-replicating swarms, space robotics stands at the intersection of our greatest technological achievements and our boldest cosmic ambitions. This evolutionary journey reflects not just engineering progress, but humanity’s expanding vision of our place in the solar system.
The transformation of space robotics spans multiple dimensions—from basic radiation-hardened components to quantum computing systems, from teleoperated lunar rovers to autonomous construction robots, and from Cold War competition to international collaborative frameworks. This progression reveals a consistent pattern: each generation of technology establishes the foundation for more capable systems while simultaneously revealing new challenges that drive further innovation. The future trajectory points toward a robotic infrastructure extending throughout our solar system, with increasingly autonomous systems working alongside humans to enable sustainable exploration and utilization of space resources.
Explore the technical specifications behind current space robotic missions, examine the emerging regulatory frameworks governing autonomous systems in space, or investigate career opportunities in this rapidly expanding field. Whether your interest lies in the advanced materials enabling these systems, the artificial intelligence guiding their operations, or the international partnerships making them possible, the evolution of space robotics offers a compelling window into humanity’s expanding cosmic presence and technological capabilities.
The SpaceQuest© Map
The SpaceQuest© map is a powerful web research tool for quick information retrieval and in-depth analysis. It's particularly useful for strategic analysis and field research, offering various functions and options to explore specific topics. Experiment with its features to find the information you need.Please click here for instructions
- ⓵ ➡ check (change?) Google search language
- ⓶ select search options from top-left box
- ⓷ zoom and pan the map with mouse wheel
- ⓸ Hold and drag the left mouse button to move the map
- ⓹ click a node to highlight connected edges, and double-click on it to do a search
- ⓺ ➡ adjust search string in the search engine results page to suit your needs